Quantum control of an optically levitated nanoparticle with a mass of just one femtogram is demonstrated in a cryogenic environment by feedback-cooling the motion of the particle to the quantum ground state.
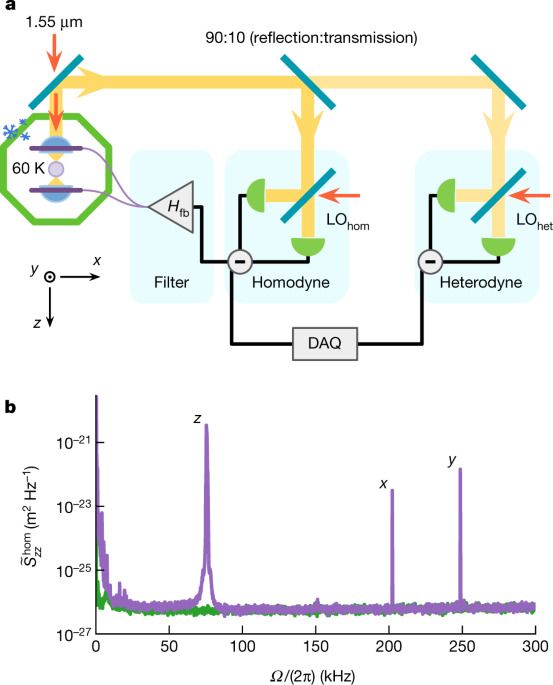
In the depths of space, there are celestial bodies where extreme conditions prevail: Rapidly rotating neutron stars generate super-strong magnetic fields. And black holes, with their enormous gravitational pull, can cause huge, energetic jets of matter to shoot out into space. An international physics team with the participation of the Helmholtz-Zentrum Dresden-Rossendorf (HZDR) has now proposed a new concept that could allow some of these extreme processes to be studied in the laboratory in the future: A special setup of two high-intensity laser beams could create conditions similar to those found near neutron stars. In the discovered process, an antimatter jet is generated and accelerated very efficiently. The experts present their concept in the journal Communications Physics.
The basis of the new concept is a tiny block of plastic, crisscrossed by micrometer-fine channels. It acts as a target for two lasers. These simultaneously fire ultra-strong pulses at the block, one from the right, the other from the left — the block is literally taken by laser pincers. “When the laser pulses penetrate the sample, each of them accelerates a cloud of extremely fast electrons,” explains HZDR physicist Toma Toncian. “These two electron clouds then race toward each other with full force, interacting with the laser propagating in the opposite direction.” The following collision is so violent that it produces an extremely large number of gamma quanta — light particles with an energy even higher than that of X-rays.
The swarm of gamma quanta is so dense that the light particles inevitably collide with each other. And then something crazy happens: According to Einstein’s famous formula E=mc2, light energy can transform into matter. In this case, mainly electron-positron pairs should be created. Positrons are the antiparticles of electrons. What makes this process special is that “very strong magnetic fields accompany it,” describes project leader Alexey Arefiev, a physicist at the University of California at San Diego. “These magnetic fields can focus the positrons into a beam and accelerate them strongly.” In numbers: Over a distance of just 50 micrometers, the particles should reach an energy of one gigaelectronvolt (GeV) — a size that usually requires a full-grown particle accelerator.
One of the most important open questions in science is how our consciousness is established. In the 1990s, long before winning the 2020 Nobel Prize in Physics for his prediction of black holes, physicist Roger Penrose teamed up with anaesthesiologist Stuart Hameroff to propose an ambitious answer.
They claimed that the brain’s neuronal system forms an intricate network and that the consciousness this produces should obey the rules of quantum mechanics —the theory that determines how tiny particles like electrons move around. This, they argue, could explain the mysterious complexity of human consciousness.
Penrose and Hameroff were met with incredulity. Quantum mechanical laws are usually only found to apply at very low temperatures. Quantum computers, for example, currently operate at around -272°C. At higher temperatures, classical mechanics takes over. Since our body works at room temperature, you would expect it to be governed by the classical laws of physics. For this reason, the quantum consciousness theory has been dismissed outright by many scientists—though others are persuaded supporters.
A novel, high-resolution fluorescence imaging technique reveals a pattern, known as a Pauli crystal, that can emerge in a cloud of trapped, noninteracting fermions.
Bring two particles together and, in general, they will interact. For example, two electrons will repel each other through electrostatic forces, or two atoms may form a molecule through electrostatic and van der Waals forces. Noninteracting particles, however, can also affect another’s behavior in a way that depends on the spin of both particles. In particular, fermionic particles, which have half-integer spin, obey the Pauli exclusion principle, which states that two fermions can never occupy the same quantum state. Two electrons in an atom, for instance, can never occupy the same quantum state. As a result, noninteracting particles can form self-organized structures. However, these structures, called Pauli crystals, have not been previously observed. Now using ultracold atoms, Marvin Holten from the University of Heidelberg, Germany, and colleagues have experimentally realized and imaged a Pauli crystal [1].
The tool next examines how one protein’s amino acids interact with another within the same protein, for example, by examining the distance between two distant building blocks. It’s like looking at your hands and feet fully stretched out, versus in a backbend measuring the distance between those extremities as you “fold” into a yoga pose.
Finally, the third track looks at 3D coordinates of each atom that makes up a protein building block—kind of like mapping the studs on a Lego block—to compile the final 3D structure. The network then bounces back and forth between these tracks, so that one output can update another track.
The end results came close to those of DeepMind’s tool, AlphaFold2, which matched the gold standard of structures obtained from experiments. Although RoseTTAFold wasn’t as accurate as AlphaFold2, it seemingly required much less time and energy. For a simple protein, the algorithm was able to solve the structure using a gaming computer in about 10 minutes.
One of the most important open questions in science is how our consciousness is established. In the 1990s, long before winning the 2020 Nobel Prize in Physics for his prediction of black holes, physicist Roger Penrose teamed up with anaesthesiologist Stuart Hameroff to propose an ambitious answer.
They claimed that the brain’s neuronal system forms an intricate network and that the consciousness this produces should obey the rules of quantum mechanics – the theory that determines how tiny particles like electrons move around. This, they argue, could explain the mysterious complexity of human consciousness.
Penrose and Hameroff were met with incredulity. Quantum mechanical laws are usually only found to apply at very low temperatures. Quantum computers, for example, currently operate at around -272°C. At higher temperatures, classical mechanics takes over. Since our body works at room temperature, you would expect it to be governed by the classical laws of physics. For this reason, the quantum consciousness theory has been dismissed outright by many scientists – though others are persuaded supporters.
Circa 1999 could lead to a sorta room temperature hydrogen fill up.
Masses of single-walled carbon nanotubes (SWNTs) with a large mean diameter of about 1.85 nanometers, synthesized by a semicontinuous hydrogen arc discharge method, were employed for hydrogen adsorption experiments in their as-prepared and pretreated states. A hydrogen storage capacity of 4.2 weight percent, or a hydrogen to carbon atom ratio of 0.52, was achieved reproducibly at room temperature under a modestly high pressure (about 10 megapascal) for a SWNT sample of about 500 milligram weight that was soaked in hydrochloric acid and then heat-treated in vacuum. Moreover, 78.3 percent of the adsorbed hydrogen (3.3 weight percent) could be released under ambient pressure at room temperature, while the release of the residual stored hydrogen (0.9 weight percent) required some heating of the sample.
Experiments now online or being built in Japan, South Korea, Italy, Canada and the United States are an order of magnitude more sensitive than the previous generation, and planned future detectors would improve on that by another two orders of magnitude (see ‘Experiments around the world’). In 2015, an advisory committee to the US Department of Energy identified such a project as a priority and a commitment to fund an experiment to detect Majorana neutrinos — estimated to cost up to/around US$250 million — is thought to be imminent.
The search for exotic ‘Majorana’ particles that could solve a big antimatter mystery is ramping up around the world.
The Juno Waves instrument “listened” to the radio emissions from Jupiter’s immense magnetic field to find their precise locations.
By listening to the rain of electrons flowing onto Jupiter from its intensely volcanic moon Io, researchers using NASA’s Juno spacecraft have found what triggers the powerful radio emissions within the monster planet’s gigantic magnetic field. The new result sheds light on the behavior of the enormous magnetic fields generated by gas-giant planets like Jupiter.
Jupiter has the largest, most powerful magnetic field of all the planets in our solar system, with a strength at its source about 20000 times stronger than Earth’s. It is buffeted by the solar wind, a stream of electrically charged particles and magnetic fields constantly blowing from the Sun. Depending on how hard the solar wind blows, Jupiter’s magnetic field can extend outward as much as two million miles (3.2 million kilometers) toward the Sun and stretch more than 600 million miles (over 965 million kilometers) away from the Sun, as far as Saturn’s orbit.